Proteins as a building block of colloidal delivery
1.1 Albumin: Albumin is a globular protein that occupies nearly 60% of the human proteome (concentration of 40mg/mL in whole blood) and has clinically proven to be important buffering material(1). The concentration of albumin reduces with age-related chronic conditions (like cancer or Alzheimer's disease) (2-4). Use of albumin (5% to 25 %w/v) solution via. intravenous route (IV) has been an important part of providing therapeutic relief for hypovolemia in chronic conditions(5, 6). This shows that maintaining a native albumin concentration of around 50g/dL and an A/G ratio >2 can provide protection against cancer(7). As biomaterial albumin helps to increase the circulation half-life of rapidly clearing drugs. It has been explored as a promising sustainable green biomaterial for drug delivery with the potential of being explored as a biomimetic system(8).
Albumin nanoparticles have been used for the delivery of various anticancer drugs (paclitaxel, luminespib (Hsp90 inhibitor), siRNA, and others) for anticancer therapies(9, 10). Paclitaxel-bound albumin nanoparticle (Abraxane), is a billion-dollar annual revenue drug and it was the first FDA-approved nanoparticle. Abraxane is a DMSO (co-solvent) -free formulation, it was developed in response to the hypersensitivity reactions caused due to the treatment with solvent-based paclitaxel infusions. Compared to solvent-based paclitaxel, albumin-bound paclitaxel allows for shorter infusion times and the eliminated need for pre-medication for hypersensitivity reactions. Albumin-bound paclitaxel has also displayed a higher amount of drug delivery to tumor cells and increased transport across endothelial cell membranes; the proposed mechanism for this phenomenon is that it can use the body's endogenous albumin pathways, including enhanced cell permeation and retention via receptor-mediated transcytosis, which allows the drug to more easily pass through endothelial cell layers for delivery at tumor cells(11). In addition, albumin nanoparticles have been developed to overcome the rapid elimination and increase delivery of the siRNA to target tissues. Materials traditionally used in nanocarriers include complex lipid and polymer formulations which can be immunogenic and cytotoxic to noncancerous cells; they can also exhibit a preferential uptake in the liver and spleen instead of the target tissues. Instead, endogenous human albumin was developed as a nanocarrier for siRNA. Unmodified siRNA does not bind to albumin so siRNA was first conjugated to a diacyl lipid moiety, then allowed to formulate with albumin. This formulation was found to have reduced renal clearance, 5.7 times longer half-life, and an 8.6 times higher bioavailability compared to unmodified siRNA. When studied in mice, the siRNA bound to albumin was compared to siRNA delivered with a commercially available carrier, jetPEI. In comparison to the jetPEI formulation, the albumin-bound siRNA resulted in a 46-fold increase in tumor cell uptake in the breast cancer mice model. Additionally, it had a significantly higher ratio of concentration in the tumor cells as opposed to the liver than jetPEI siRNA(12).
Albunex microbubbles were developed, which provides an example that albumin can also be used as an ultrasound contrast agent(13). This shows the application of albumin as biomaterial for developing theranostic agent. Later in the 1990s, it was discovered that albumin is a protein capable of crosslinking via side-chain interactions when heat is applied in solution; this thermal denaturation process can result in an albumin gel(14). Our group also experimented to understand the biomimetic viscoelastic nature of the albumin gels compared to human tissue in order to evaluate the capabilities of physically crosslinked albumin hydrogels. The structure of the gel is dependent on several factors, including heating and duration of heating, and the protein aggregation pattern that is strongly determined by pH and salt content. Additionally, alteration of the salt concentrations or type of salts in the albumin solution or the duration or temperature during the heating process, the stiffness of the gel can be altered, creating densities more consistent with different body tissues(14). A group of young chemists also demonstrated that simple and controlled dehydration of albumin gels can lead to the development of flexible clear films through molecular aggregation followed by controlled dehydration(15). These formulation variations are attributed to changes in the overall charge and confirmation of the albumin protein. The isoelectric point (PI) of albumin is around 5.1 and exhibits a negative charge at physiological pH (~ 7.4)(16). Whereas, at acidic pH, the net charge of the system can be positive. Hence, ligand-protein interaction can be altered depending on the pH of the matrix and can aid in improved nano-formulations. Moreover, some studies also suggest that conformational states of the albumin also change with pH(17). Hence, the changes in conformational states of albumin in presence of salts, and pH can help change the viscoelastic nature of the biomimetic hydrogels. However, most of this data is only spectroscopy determinations no structural confirmation has been provided for this science. This makes the material one of the most important biomaterials of the decade for being explored in the development of biomimetic nano therapies(18, 19). The commonly explored source of this material is either human or bovine (Table 1) making it a sustainable source for developing efficient deliver systems.
1.2 Collagen: Collagen is a fiber-like protein structure that forms connective tissues. Collagen is categorized into 28 members (Type I- XXVIII) with Type I being the strongest and most abundant protein found in the body(20). Similar to albumin, collagen levels in the body drop with age and other life activities such as smoking, alcohol, lack of sleep, exercise, and others(21). Collagen fibrils act as a resisting composite system of the ECM. It is a non-cellular component within all tissues and organs. Some studies have also shown that collagen is also the source of natural gelatin. Collagen is considered a safe and effective biomaterial in both clinical applications and tissue engineering. For instance, it is included in products in dental composites, biodegradable matrices, skin regeneration templates, cardiovascular and plastic surgery, orthopedics, urology, neurology, and ophthalmology. Collagen has been studied to be used in the manufacture of supplements that have been increasingly used and advertised as an antiaging remedy(22-24). As mentioned earlier, aging causes a reduction of collagen that also causes the skin to lose functionality of the skin. Aging has a significant effect on the connective tissue of the skin and results in the decline in elastin and collagen fibers leading to wrinkles. Collagen is the main material used in manufacturing several hydrogels which are used in beauty masks. This restores the elasticity of the skin and promotes anti-aging performance(25). Collagen fibers recommended by experts are essentially increasing moisture retention in the skin. Hence, from a commercial perspective, it makes collagen one of the most explored materials in the cosmetic industry.
The loss of collagen type II contributed to chondrocyte hypertrophy via. the bone morphogenetic protein (BPM)- SMAD1 pathway(26). This result demonstrates the mechanism of chondrocyte hypertrophy; hence inhibition of collagen type II potentially initiates the progression of osteoarthritis. Collagen-II was proven to be an important signaling molecule in the regulation of chondrocyte proliferation, differentiation, and metabolism(27), and its deficiency caused potential chondrocyte hypertrophy in osteoarthritis cartilage. This is a potential cause of osteoarthritis. Preclinical and clinical evaluations of treatment with type II collagen show significant improvement in patients with osteoarthritis(28). A plastically compressed dense collagen (DC) gel system have been studied to dually support seed cell growth(normal human fibroblast), which provides a highly potent osteoid-like environment for nucleation and growth of crystalline hydroxyapatite (HA)(29). Collagen is easily degradable through extracellular collagenase which promotes tissue regeneration when absorbed at the injury sites on the body and can therefore be used for biomaterial fabrication(30). Collagen thus promotes wound healing by enhancing collagen chemotactic on the cells. Regular collagen that is reduced to the nanometer size (1-100 nm) has the potential to be a 3D biomaterial, which provides a high surface area-to-volume ratio, which can effectively penetrate the wound site and promote healing(31). Type I and Type II collagens are explored for making gels in native and composite states (with PVA, hyaluronic acid, and other materials) to make biomimetic systems for localized application(32-34). Collagen gels can be prepared through chemical crosslinking (using glutaraldehyde and transglutaminase) or natural physical gelation mechanisms(35-37).
Cancer cells can also participate in the synthesis of ECM and cancer associate fibroblasts are the main source of collagen in tumors. The processes of collagen biosynthesis and remodeling in parallel were studied with the transcriptome dynamics during cancer cells and fibroblasts interaction(38). Studies have shown that, highly hydrated (HH) type I collagen hydrogels provide a sufficient platform for solid tumor development using MDA-MB-231 cells. Necrosis and vascularization/angiogenesis (VA) markers were studied to be up-regulated in cancer cells at a specific depth between 150 µm and 200 µm within the gels(39). An injectable drug system specially developed to treat local tumors to achieve high, sustained, and homogenous intra-tumoral drug concentrations without toxicity(40). Evidence suggests that cancer cells somehow like collagen material and can be 3D bioprinted that provides an interesting opportunity to explore this material as a biomimetic delivery system(41, 42).
3. Polysaccharide formulation structures for biomimetic delivery:
Hyaluronic acid (HA) is the second most commonly explored material from ECM in pharmaceutical sciences. Chemically the material is a polysaccharide (repeating disaccharide units of glucuronic acid and N- acetylglucosamine) and is most abundantly found in skin and other body tissues. It was first isolated in 1934 by scientists Meyer and Palmer. The first pharmaceutical-grade HA was developed in 1979 by Balazs' who invented an efficient procedure to extract HA from rooster combs and human umbilical cords. HA is found to be beneficial for the treatment of skin disease, wound healing, tissue repair, lubrication of the joints, soft tissue augmentation, and the prevention and treatment of extrinsic skin aging. In recent years, hyaluronic acid has been utilized as an “ideal” facial filler(43). Before the 1980s and 1990's US physicians were contented to use collagen-based injectables. However, in recent years hyaluronic acid fillers have become increasingly popular due to their hydrophilicity-mediated capacity to retain water. HA in its native and composite state with corn silk extract has been explored as a biomimetic composite scaffold for regenerative medicine for bone tissue regeneration(44). Furthermore, studies have demonstrated that hyaluronic acid may be utilized for the treatment of osteoarthritis of the knee. In a recent study, the data concluded that patients had a significant reduction in knee pain with an HA injection vs. a placebo(45-47).
From a nano-formulation perspective, HA-based nanoparticles have effective means to deliver cancer therapies. These therapies include drug-conjugated HA micelles, polymersomes, and hydrogels. HA nanomaterials are non-inflammatory, biodegradable, do not provoke an immune response, and have great biocompatibility. HA may be directly conjugated to anticancer drugs to deliver antitumor effects. Because HA-targeted receptors like CD44, are overexpressed in cancer cells, drug-conjugated HA complexes improve treatment efficacy and specifically target cancer cells(48). Drug-conjugated HA additionally provides the benefits of increased drug stability, increased solubility, cancer-targeting abilities, and increased circulation time(49). Additionally, HA micelles result in amphiphilic nanoparticles that can effectively deliver hydrophobic drugs to target cancer cells. Injectable HA-based hydrogels loaded with paclitaxel (PTX) and epirubicin (EPB) has been shown to steadily release drugs to prevent tumor recurrence and lead to prolonged survival in a post-operative breast tumor model(50). No systemic toxicities were observed, and proliferation and migration of in vitro tumor cells were inhibited. Because of hyaluronic acid's versatility and biocompatibility, it appears that HA has a promising future in biomimetic applications. Availability of HA from plant, animals and microbial sources makes it sustainable in nature (Table 1).
2. DNA origami and gels
DNA is the carrier of genetic information; it deals with the function and development of an organism. DNA is self-replicating and is present in all organisms. DNA was recognized as the smartest biomaterial due to its characteristic feature of self-assembly, making it a suitable biomaterial for various biomedical applications. Origami of the DNA is the nanoscale folding of the DNA molecule to create two and three –dimensional shapes at the nanoscale(51). DNA origami is used to create three-dimensional structures, that are useful for designing an effective drug delivery system. They can be used as drug delivery vehicles to transport therapeutic agents into the cell. DNA origami is a promising approach for drug delivery systems, but one of the challenges that were faced during application was immune system activation. To overcome this problem, a compact short-tubular DNA origami (STDO) was designed and synthesized. STDO was placed in doxorubicin-loaded stealth liposomes without a pH-driven gradient. This application will open the door to encapsulating many other hydrophobic drugs(52). This technology allows the construction of nanoscale containers, a promising approach for creating biomimetic structures(53).
Biomimetic channels derived from DNA origami have also been designed to resemble natural transmembrane channels manipulated by membrane proteins. DNA scaffolds are also powerful analogs of the cytoskeleton, guiding the formation of artificial cellular structures.
Another use of DNA as a biomedical material is the synthesis of hydrogels from DNA. These hydrogels are characterized by being biocompatible, stable, and versatile. We have 2 types of DNA hydrogels a) hybridized gels that are formed due to cross-linking in the DNA molecules and b) native DNA that is assembled by enzymatic ligation, polymerization, and specific binding of DNA motifs as building blocks(54). The programmability of DNA is exploited to achieve control over the rheology of hydrogels with temperature-regulated viscous behavior. Microrheology was used to study the mechanical properties of DNA hydrogels at the microscopic scale, and the viscoelastic response was mapped over a wide range of frequencies and temperatures. This was done to engineer DNA-based materials with precise control of structural stability and stiffness at the molecular level(55). Smart DNA hydrogels are types of DNA hydrogels that show a reversible state (hydrogel to solution, or hydrogel to solid) under different triggers like pH, temperature, ionic strength, and electric field strength, this level of control in mechanical properties will help design more controlled release systems(54).
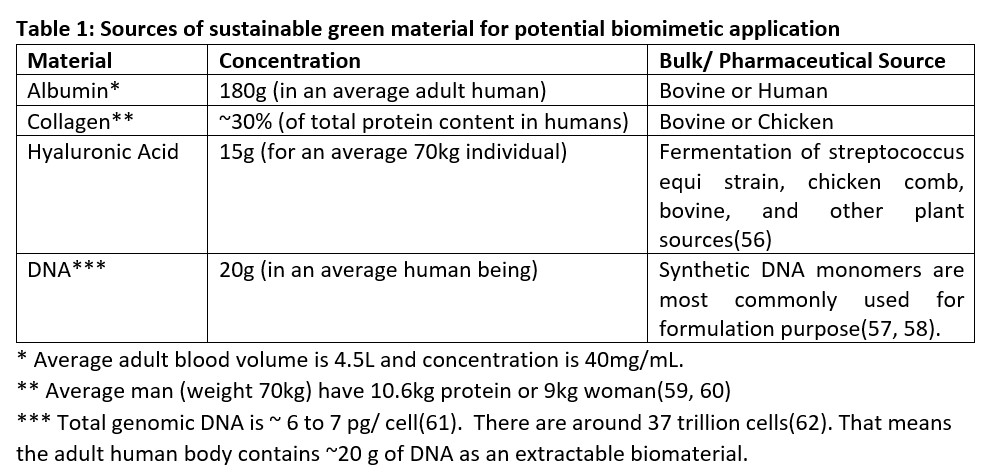
Figure 1: Schematic representation of the source of sustainable green biomaterials like albumin, collagen, hyaluronic acid, and DNA. Various possible formulations ((a) hydrogel, (b) printable gels, (c) nanofibers, (d) nanoparticles and microbubbles) are possible from materials in the native and composite state.
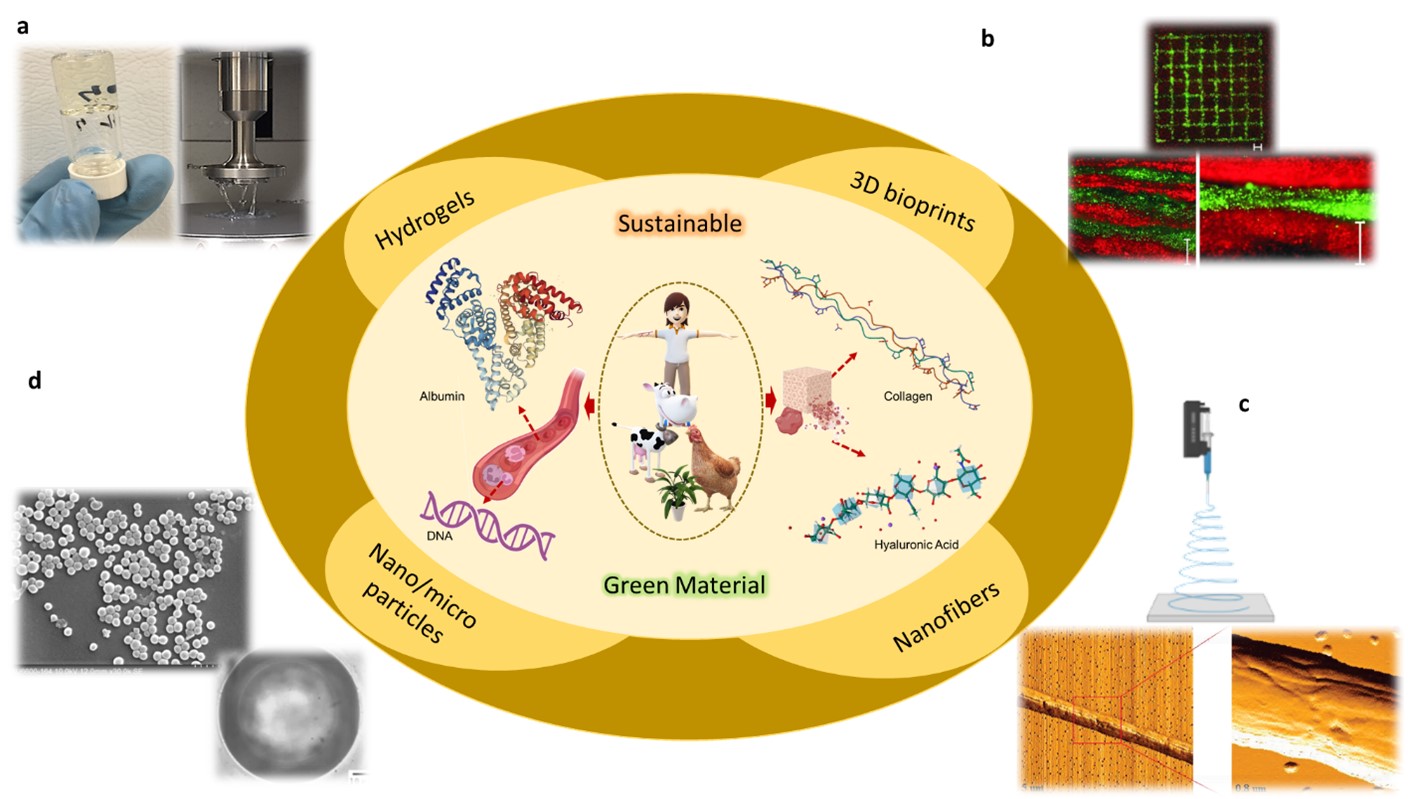
References:
1. Ezra A, Rabinovich-Nikitin I, Rabinovich-Toidman P, Solomon B. Chapter 11 - Multifunctional Effects of Human Serum Albumin Toward Neuroprotection in Alzheimer Disease. In: Gozes I, editor. Neuroprotection in Alzheimer's Disease: Academic Press; 2017. p. 217-38.
2. Kim JW, Byun MS, Lee JH, Yi D, Jeon SY, Sohn BK, et al. Serum albumin and beta-amyloid deposition in the human brain. Neurology. 2020;95(7):e815-e26.
3. Menendez-Gonzalez M, Gasparovic C. Albumin Exchange in Alzheimer's Disease: Might CSF Be an Alternative Route to Plasma? Front Neurol. 2019;10:1036.
4. Gupta D, Lis CG. Pretreatment serum albumin as a predictor of cancer survival: a systematic review of the epidemiological literature. Nutr J. 2010;9:69.
5. Vincent JL, Russell JA, Jacob M, Martin G, Guidet B, Wernerman J, et al. Albumin administration in the acutely ill: what is new and where next? Crit Care. 2014;18(4):231.
6. Yu YT, Liu J, Hu B, Wang RL, Yang XH, Shang XL, et al. Expert consensus on the use of human serum albumin in critically ill patients. Chin Med J (Engl). 2021;134(14):1639-54.
7. Seaton K. Albumin concentration controls cancer. J Natl Med Assoc. 2001;93(12):490-3.
8. Hoogenboezem EN, Duvall CL. Harnessing albumin as a carrier for cancer therapies. Adv Drug Deliv Rev. 2018;130:73-89.
9. Ma P, Mumper RJ. Paclitaxel Nano-Delivery Systems: A Comprehensive Review. J Nanomed Nanotechnol. 2013;4(2):1000164.
10. A Rochani, Balasubramanian S, Ravindran Girija A, Maekawa T, Kaushal G, Kumar DS. Heat Shock Protein 90 (Hsp90)-Inhibitor-Luminespib-Loaded-Protein-Based Nanoformulation for Cancer Therapy. Polymers (Basel). 2020;12(8).
11. Kundranda MN, Niu J. Albumin-bound paclitaxel in solid tumors: clinical development and future directions. Drug Des Devel Ther. 2015;9:3767-77.
12. Sarett SM, Werfel TA, Lee L, Jackson MA, Kilchrist KV, Brantley-Sieders D, et al. Lipophilic siRNA targets albumin in situ and promotes bioavailability, tumor penetration, and carrier-free gene silencing. Proc Natl Acad Sci U S A. 2017;114(32):E6490-E7.
13. Ultrasound contrast agents. In: Aronson JK, editor. Meyler's Side Effects of Drugs (Sixteenth Edition). Oxford: Elsevier; 2016. p. 244-7.
14. Murata M, Tani F, Higasa T, Kitabatake N, Doi E. Heat-induced Transparent Gel Formation of Bovine Serum Albumin. Bioscience, Biotechnology, and Biochemistry. 1993;57(1):43-6.
15. Yamazoe H, Tanabe T. Preparation of water-insoluble albumin film possessing nonadherent surface for cells and ligand binding ability. J Biomed Mater Res A. 2008;86(1):228-34.
16. Fologea D, Ledden B, McNabb DS, Li J. Electrical characterization of protein molecules by a solid-state nanopore. Appl Phys Lett. 2007;91(5):539011-3.
17. Baler K, Martin OA, Carignano MA, Ameer GA, Vila JA, Szleifer I. Electrostatic unfolding and interactions of albumin driven by pH changes: a molecular dynamics study. J Phys Chem B. 2014;118(4):921-30.
18. Liu L, Bi Y, Zhou M, Chen X, He X, Zhang Y, et al. Biomimetic Human Serum Albumin Nanoparticle for Efficiently Targeting Therapy to Metastatic Breast Cancers. ACS Appl Mater Interfaces. 2017;9(8):7424-35.
19. Lin T, Zhao P, Jiang Y, Tang Y, Jin H, Pan Z, et al. Blood-Brain-Barrier-Penetrating Albumin Nanoparticles for Biomimetic Drug Delivery via Albumin-Binding Protein Pathways for Antiglioma Therapy. ACS Nano. 2016;10(11):9999-10012.
20. Ricard-Blum S. The collagen family. Cold Spring Harb Perspect Biol. 2011;3(1):a004978.
21. The Nutrition Source 2023
22. Holmes DF, Lu Y, Starborg T, Kadler KE. Collagen Fibril Assembly and Function. Curr Top Dev Biol. 2018;130:107-42.
23. Al-Atif H. Collagen Supplements for Aging and Wrinkles: A Paradigm Shift in the Fields of Dermatology and Cosmetics. Dermatol Pract Concept. 2022;12(1):e2022018.
24. Zhao X, Zhang X, Liu D. Collagen peptides and the related synthetic peptides: A review on improving skin health. Journal of Functional Foods. 2021;86:104680.
25. Bolke L, Schlippe G, Gerss J, Voss W. A Collagen Supplement Improves Skin Hydration, Elasticity, Roughness, and Density: Results of a Randomized, Placebo-Controlled, Blind Study. Nutrients. 2019;11(10).
26. Lian C, Wang X, Qiu X, Wu Z, Gao B, Liu L, et al. Collagen type II suppresses articular chondrocyte hypertrophy and osteoarthritis progression by promoting integrin beta1-SMAD1 interaction. Bone Res. 2019;7:8.
27. Wu Z, Korntner SH, Mullen AM, Zeugolis DI. Collagen type II: From biosynthesis to advanced biomaterials for cartilage engineering. Biomaterials and Biosystems. 2021;4:100030.
28. Crowley DC, Lau FC, Sharma P, Evans M, Guthrie N, Bagchi M, et al. Safety and efficacy of undenatured type II collagen in the treatment of osteoarthritis of the knee: a clinical trial. Int J Med Sci. 2009;6(6):312-21.
29. Brown RA, Wiseman M, Chuo CB, Cheema U, Nazhat SN. Ultrarapid Engineering of Biomimetic Materials and Tissues: Fabrication of Nano- and Microstructures by Plastic Compression. Advanced Functional Materials. 2005;15(11):1762-70.
30. An YZ, Kim YK, Lim SM, Heo YK, Kwon MK, Cha JK, et al. Physiochemical properties and resorption progress of porcine skin-derived collagen membranes: In vitro and in vivo analysis. Dent Mater J. 2018;37(2):332-40.
31. Naskar A, Kim KS. Recent Advances in Nanomaterial-Based Wound-Healing Therapeutics. Pharmaceutics. 2020;12(6).
32. Lan W, Xu M, Zhang X, Zhao L, Huang D, Wei X, et al. Biomimetic polyvinyl alcohol/type II collagen hydrogels for cartilage tissue engineering. Journal of Biomaterials Science, Polymer Edition. 2020;31(9):1179-98.
33. Puttawibul P, Meesane J, Benjakul S, editors. Preparation and characterization of type I collagen/PVA hybrid biomimetic hydrogels scaffold for wound healing. The 5th 2012 Biomedical Engineering International Conference; 2012 5-7 Dec. 2012.
34. Marin S, Albu Kaya MG, Ghica MV, Dinu-Pirvu C, Popa L, Udeanu DI, et al. Collagen-Polyvinyl Alcohol-Indomethacin Biohybrid Matrices as Wound Dressings. Pharmaceutics. 2018;10(4).
35. Chuang CH, Lin RZ, Melero-Martin JM, Chen YC. Comparison of covalently and physically cross-linked collagen hydrogels on mediating vascular network formation for engineering adipose tissue. Artif Cells Nanomed Biotechnol. 2018;46(sup3):S434-S47.
36. Orban JM, Wilson LB, Kofroth JA, El-Kurdi MS, Maul TM, Vorp DA. Crosslinking of collagen gels by transglutaminase. J Biomed Mater Res A. 2004;68(4):756-62.
37. O Halloran DM, Collighan RJ, Griffin M, Pandit AS. Characterization of a Microbial Transglutaminase Cross-linked Type II Collagen Scaffold. Tissue Engineering. 2006;12(6):1467-74.
38. Druzhkova I, Shirmanova M, Ignatova N, Dudenkova V, Lukina M, Zagaynova E, et al. Expression of EMT-Related Genes in Hybrid E/M Colorectal Cancer Cells Determines Fibroblast Activation and Collagen Remodeling. Int J Mol Sci. 2020;21(21).
39. Szot CS, Buchanan CF, Freeman JW, Rylander MN. 3D in vitro bioengineered tumors based on collagen I hydrogels. Biomaterials. 2011;32(31):7905-12.
40. Orenberg EK. Intralesional Chemotherapy with Injectable Collagen Gel Formulations. In: Brown DM, editor. Drug Delivery Systems in Cancer Therapy. Totowa, NJ: Humana Press; 2004. p. 229-46.
41. Osidak EO, Kozhukhov VI, Osidak MS, Domogatsky SP. Collagen as Bioink for Bioprinting: A Comprehensive Review. Int J Bioprint. 2020;6(3):270.
42. Hsu KS, Dunleavey JM, Szot C, Yang L, Hilton MB, Morris K, et al. Cancer cell survival depends on collagen uptake into tumor-associated stroma. Nat Commun. 2022;13(1):7078.
43. Fallacara A, Baldini E, Manfredini S, Vertuani S. Hyaluronic Acid in the Third Millennium. Polymers (Basel). 2018;10(7).
44. Makvandi P, Ali GW, Della Sala F, Abdel-Fattah WI, Borzacchiello A. Hyaluronic acid/corn silk extract based injectable nanocomposite: A biomimetic antibacterial scaffold for bone tissue regeneration. Mater Sci Eng C Mater Biol Appl. 2020;107:110195.
45. Migliore A, Blicharski T, Plebanski R, Zegota Z, Gyula G, Rannou F, et al. Knee Osteoarthritis Pain Management with an Innovative High and Low Molecular Weight Hyaluronic Acid Formulation (HA-HL): A Randomized Clinical Trial. Rheumatol Ther. 2021;8(4):1617-36.
46. Huang TL, Chang CC, Lee CH, Chen SC, Lai CH, Tsai CL. Intra-articular injections of sodium hyaluronate (Hyalgan(R)) in osteoarthritis of the knee. a randomized, controlled, double-blind, multicenter trial in the Asian population. BMC Musculoskelet Disord. 2011;12:221.
47. Strand V, Conaghan PG, Lohmander LS, Koutsoukos AD, Hurley FL, Bird H, et al. An integrated analysis of five double-blind, randomized controlled trials evaluating the safety and efficacy of a hyaluronan product for intra-articular injection in osteoarthritis of the knee. Osteoarthritis Cartilage. 2006;14(9):859-66.
48. Kesharwani P, Chadar R, Sheikh A, Rizg WY, Safhi AY. CD44-Targeted Nanocarrier for Cancer Therapy. Front Pharmacol. 2021;12:800481.
49. Kim JH, Moon MJ, Kim DY, Heo SH, Jeong YY. Hyaluronic Acid-Based Nanomaterials for Cancer Therapy. Polymers (Basel). 2018;10(10).
50. Leng Q, Li Y, Zhou P, Xiong K, Lu Y, Cui Y, et al. Injectable hydrogel loaded with paclitaxel and epirubicin to prevent postoperative recurrence and metastasis of breast cancer. Mater Sci Eng C Mater Biol Appl. 2021;129:112390.
51. Rochani AK. RS, Kumar DS. Sustainable Green Polymeric Nanoconstructs for Active and Passive Cancer Therapeutics: Jenny Stanford Publishing; 2020.
52. Palazzolo S, Hadla M, Spena CR, Bayda S, Kumar V, Lo Re F, et al. Proof-of-Concept Multistage Biomimetic Liposomal DNA Origami Nanosystem for the Remote Loading of Doxorubicin. ACS Med Chem Lett. 2019;10(4):517-21.
53. Shen H, Wang Y, Wang J, Li Z, Yuan Q. Emerging Biomimetic Applications of DNA Nanotechnology. ACS Appl Mater Interfaces. 2019;11(15):13859-73.
54. Chen M, Wang Y, Zhang J, Peng Y, Li S, Han D, et al. Stimuli-responsive DNA-based hydrogels for biosensing applications. J Nanobiotechnology. 2022;20(1):40.
55. Xing Z, Caciagli A, Cao T, Stoev I, Zupkauskas M, O'Neill T, et al. Microrheology of DNA hydrogels. Proc Natl Acad Sci U S A. 2018;115(32):8137-42.
56. Gupta RC, Lall R, Srivastava A, Sinha A. Hyaluronic Acid: Molecular Mechanisms and Therapeutic Trajectory. Front Vet Sci. 2019;6:192.
57. Um SH, Lee JB, Park N, Kwon SY, Umbach CC, Luo D. Enzyme-catalysed assembly of DNA hydrogel. Nat Mater. 2006;5(10):797-801.
58. Lattuada E, Leo M, Caprara D, Salvatori L, Stoppacciaro A, Sciortino F, et al. DNA-GEL, Novel Nanomaterial for Biomedical Applications and Delivery of Bioactive Molecules. Front Pharmacol. 2020;11:01345.
59. Wang Z, Shen W, Kotler DP, Heshka S, Wielopolski L, Aloia JF, et al. Total body protein: a new cellular level mass and distribution prediction model. The American Journal of Clinical Nutrition. 2003;78(5):979-84.
60. Duda K, Majerczak J, Nieckarz Z, Heymsfield SB, Zoladz JA. Chapter 1 - Human Body Composition and Muscle Mass. In: Zoladz JA, editor. Muscle and Exercise Physiology: Academic Press; 2019. p. 3-26.
61. Wang F, Pan X, Kalmbach K, Seth-Smith ML, Ye X, Antumes DM, et al. Robust measurement of telomere length in single cells. Proc Natl Acad Sci U S A. 2013;110(21):E1906-12.
62. Roy AL, Conroy RS. Toward mapping the human body at a cellular resolution. Mol Biol Cell. 2018;29(15):1779-85.